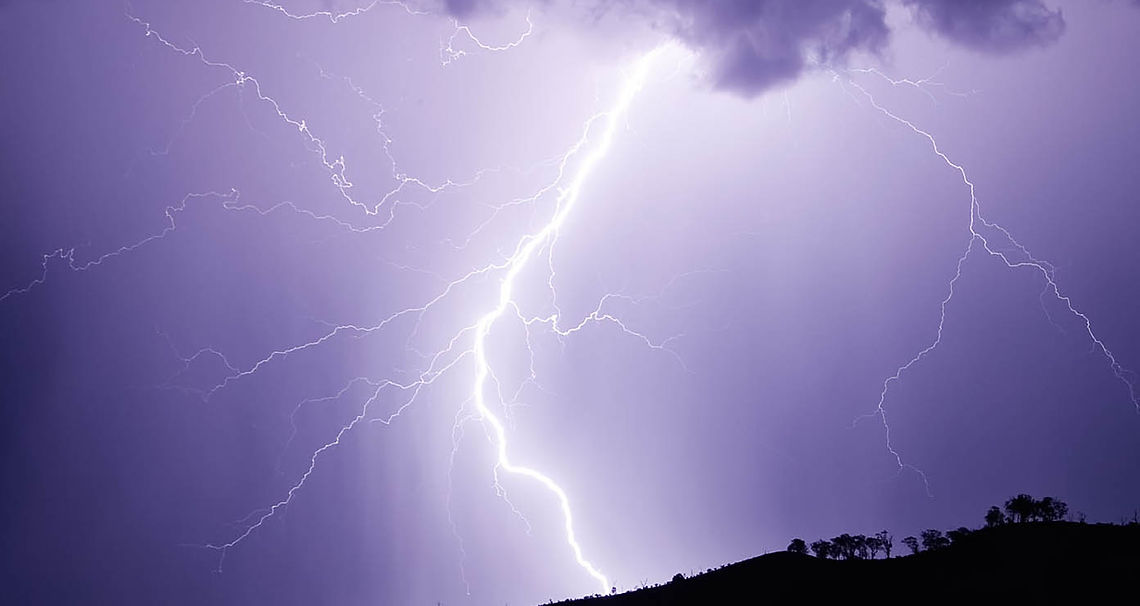
PUZZLES FROM THE DYNAMIC UNIVERSE
(This site is no longer being updated)
Recent years have seen the opening of many new windows in the time, wavelength, and messenger domains, which led to the discovery of many energetic transient phenomena,
including intense radio bursts of unknown origin from random directions in the sky, bright multi-wavelength radiation produced when a star is torn apart by a black hole, gravitational waves from mergers of neutron stars/black holes, and rare supernovae more than ten times more luminous than their commonly known cousins. I am broadly interested in understanding the physical processes in these transients as well as extracting the encoded information about the evolution of massive stars, physics of compact objects, and demographics of supermassive black holes.
Wenbin's website has permanently moved to:
https://wenbinlu.github.io
EDUCATION
I will be joining University of California Berkeley as an Assistant Professor in 2022.
I am currently a Lyman Spitzer Fellow at Princeton University. I was a Burke Fellow at the California Institute of Technology (2018-2021).
I received my PhD degree in Astronomy at the University of Texas at Austin in 2018, under the supervision of Prof. Pawan Kumar.
I received my bachelor's degree in physics from Yuanpei College, Peking University (PKU) in 2013. The Yuanpei Program, named after PKU’s most revered president, Mr. Cai Yuanpei, allows students to freely choose their field of study. I got interested in astrophysics from a coincidental discussion on cosmic rays with Prof. Zhuo Li, who later became my undergrad advisor.
RESEARCH INTERESTS
My research goal is to understand underlying physics behind various high-energy transient phenomena, including fast radio bursts (FRBs), tidal disruption events (TDEs), and compact object mergers. I have also worked on gamma-ray bursts, formation history of binary black holes, accretion disks, pre-supernova mass loss, and hyper-velocity stars. Based on my expertise in plasma physics, special/general relativity, hydrodynamics, radiative transfer, and stellar evolution, I have developed analytical and numerical methods to recognize the mechanisms responsible for the diverse observational behaviors of these transient sources.
I enjoy interactions with other researchers on various topics in astrophysics. Many of my projects were initiated during the discussions with postdocs and students. Below I describe a selection of my works. Interesting predictions from my research and observational tests are summarized in a table at the end.
-
FAST RADIO BURSTS
-
Emission Mechanism: In Lu, Kumar & Zhang (2020), we propose that FRBs are generated by Alfven waves propagating in the neutron star (NS) magnetosphere to large distances where they become charge starved, and charge clumps are accelerated by an electric field and coherently generate curvature emission in the radio band.
Figure to the Right: Alfven waves launched from the magnetic foot points propagate along the field lines to distances much larger than the NS radius where the charge density is too low to sustain the current associated with the wave. As a result of charge starvation, a strong electric field component parallel to the background B-field develops, and charge clumps are accelerated to high Lorentz factors and coherently produce curvature emission in the radio band. The FRB emission is narrowly beamed into the region spanned by the orange arrow. The Alfven waves launched far from the poles are trapped by field lines that do not extend to large distances, and a pair fireball is formed which emits hard X-rays visible from a large fraction of the sky. This model provides a unified picture for faint bursts like FRB 200428 as well as the bright events seen at cosmological distances.

Figure to the Right: Sudden magnetic energy release excite horizontal shear mode oscillations in the crust. The shear waves propagate along the crust, and the horizontal shear oscillation is well coupled to the magnetospheric B-field lines which are anchored on the NS surface. Thus, a large fraction of the shear-wave energy to be transmitted into the magnetosphere as Alfven waves.
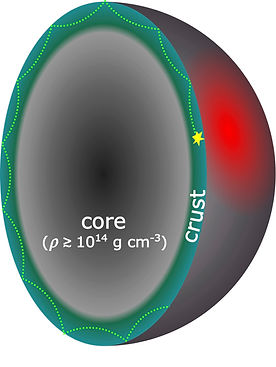
-
TIDAL DISRUPTION EVENTS
Main-sequence stars are shredded by tidal forces when they get sufficiently close to a supermassive black hole (BH). After disruption, the star is stretched into a long thin stream (of aspect ratio ~1000) in highly eccentric orbits (1-e ~ 0.01) which undergo general relativistic precession. Such precession causes the stream to self-intersect and the shocked gas expanding from the intersection point will eventually form an accretion disk, which powers multi-wavelength emission. A few dozen of these tidal disruption event (TDE) candidates have been found in recent surveys designed to find variable sources in the optical/UV and soft X-rays.
Global numerical simulation of the hydrodynamic
evolution of the thin fallback stream is prohibitively
expensive. In Lu & Bonnerot (2020), we made a key
simplification of the problem by first calculating the
location where the stream self-intersects according to
general relativistic geodesic motion and then performing
hydrodynamic simulations of the stream collision
process in a local corotating frame near the intersection
point. The two stages are joint together by a Lorentz
transformation. We found that the self-crossing shock redistributes energy and angular momentum of the gas in the fall-back stream. For sufficiently deeply penetrating orbits (Rp >~ 15Rg), the shocks at the intersection are able to unbind a large fraction (up to 50%) of the fallback gas. We call the unbound gas the “collision-induced outflow” or CIO. We found that the CIO covers a large fraction of the sky viewed from the BH and is able to reprocess the hard (EUV/soft X-ray) photons from the disk into the optical band. This provides an explanation of the bright optical emission seen in many TDEs. Viewing angle effects then cause the X-ray luminosity to vary by orders of magnitude from one event to another. The CIO carries a large kinetic energy of 1e50-1e52 erg and can generate radio emission at the level of ASASSN-14li when it drives a shock into the surrounding medium on parsec scales.
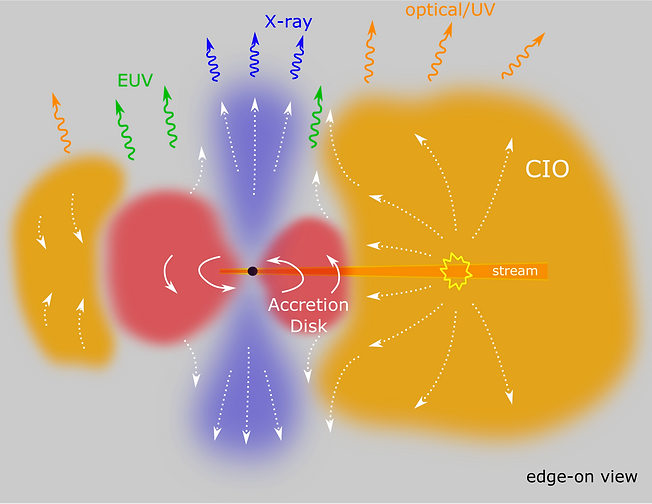
-
FORMATION OF BBH
The LIGO-Virgo Collaboration recently reported a puzzling event, GW190814, with component masses of 23 and 2.6 Msun. In Lu, Beniamini & Bonnerot (2021), we propose a 2nd-generation merger scenario where the secondary of GW190814 was from a previous binary neutron star (bNS) coalescence and the remnant was able to merge again with the 23 Msun BH tertiary. This occurs when the remnant (most likely a low-mass BH) receives a kick of about 100 km/s in the direction of the "loss cone" shaped like a flying saucer, as shown in the figure below. This model was motivated by the relatively small rate (1 to 23 per Gpc^3 per yr) inferred from GW190814 and the secondary mass being close to the total masses of known bNS systems. We show that about 1% of the bNS coalescence occurring in triple systems should give rise to 2nd-generation mergers, provided that a massive BH tertiary is located at a separation less than a few AU. Since the total bNS coalescence rate is of the order 10^3 per Gpc^3 per yr, this model requires that at least 10% bNS mergers occur in triple systems. Since the typical delay time for the 2nd-generation merger is about a Hubble time, these bNS mergers in triples occurred in the distant past when the Universe was less metal-enriched. Low-metallicity (< 0.1 solar) is also favored for the formation of the 23 Msun BH.
This model has a number of testable predictions, one of which is that the secondary of GW190814-like events should have dimensionless spin of about 0.7. Our work provides a strong motivation for future studies of triple massive star evolution.

-
GAMMA-RAY BURSTS
The classical problem of a relativistic jet interacting with the circum-stellar medium (CSM) requires expensive 2D simulations (for the axisymmetric case). In Lu, Beniamini & McDowell (2020), we present a simplified model by assuming that the shocked CSM and jet material are in an infinitely thin surface, so the original 2D problem is effectively reduced to 1D. From general conservation laws, we derive the equation of motion for each fluid element on this surface, taking into account the deceleration along the surface normal due to newly swept-up mass and lateral expansion due to pressure gradient in the tangential direction. The pressure and energy density of the shocked CSM are given by the jump conditions at the forward shock. The method is implemented with a finite-differencing method, in a new (C++) code Jedi (for “jet dynamics”). In a few seconds on a single CPU core, the code solves the jet evolution from ultra-relativistic initial conditions to non-relativistic speeds, as well as the synchrotron flux at arbitrary viewing angles and frequencies including self-absorption. It has been demonstrated in a number of test cases that our method provides a good approximation for the hydrodynamics and afterglow emission for a wide variety of jet structures and CSM density profiles.
The following figures show the lightcurves at 10^15 Hz (left panel) and 10^9 Hz (right panel) for different viewing angles (1 to 90 degrees). The solid lines include the contributions from both the forward and the counter jets, and the dashed lines are only for the counter jet (which moves away from the observer). The dotted lines are for the same initial conditions but without including the effect of lateral expansion.
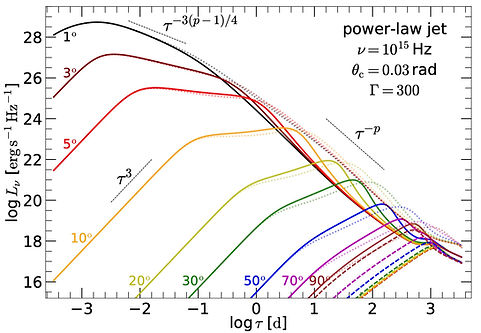
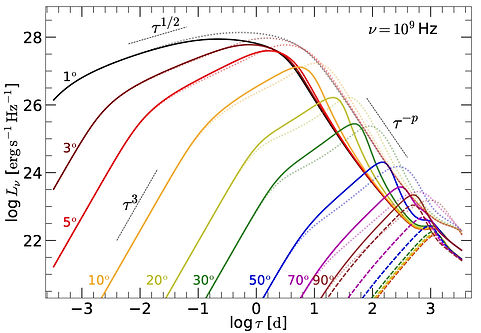
The following figure shows the hydrodynamic evolution of a power-law structured jet, with a jet core size , and energy per solid angle and four-speed beyond the jet core. The jet core has Lorentz factor 300, isotropic energy 10^52 erg, and half opening angle 0.03 rad (1.7 degree). The CSM has uniform density of 0.01 cm^-3. The jet is axisymmetric with its axis is along the x direction. The length unit is the deceleration radius. The colored lines shows the location of the jet surface at different times which are in units of the deceleration time. The black dots show the numerical grid points and the black lines are their trajectories (lateral expansion is clearly seen).




-
Interesting Predictions from my Research
(More observational tests are highly encouraged!)
PREDICTIONS
(ADS links to Publications)
Observational Tests
(links to papers)
(6) The polarization angle time series from a
repeating FRB source should be periodic [P]
not yet
(9) Most TDEs hosted by low-mass SMBHs (<10^6 Msun) should be X-ray bright but optically dim [P]
not yet
(10) Optically bright TDEs should have a shallow spectrum of Lv ~ v^0.5 in the near-IR [P]
not yet
(11) UV/optical transients from tidal disruption of
main-sequence stars by stellar-mass BHs [P]
not yet
(12) The γ/X-ray spectrum of jetted TDEs
should show a cutoff above a few MeV [P]
not yet
(13) Companion of the fastest hyper-velocity stars was captured by SgrA* in relativistic orbits [P]
not yet
(14) Infrared dust echo from binary neutron star mergers detectable by JWST [P]
not yet
-
Polarization: In Lu, Kumar & Narayan (2019), we study the propagation of FRB waves through the magnetosphere of a strongly magnetized NS. We show that the observed electric (polarization) vector is determined by the B-field orientation at the polarization-limiting radius located far from the NS surface where the dipolar field dominates (as shown in the figure below), independent of magnetic configuration of the emission region which is much closer by. Therefore, the polarization angles of repeating bursts should be periodic as modulated by the NS spin, even if the burst occurrence may not be periodic.

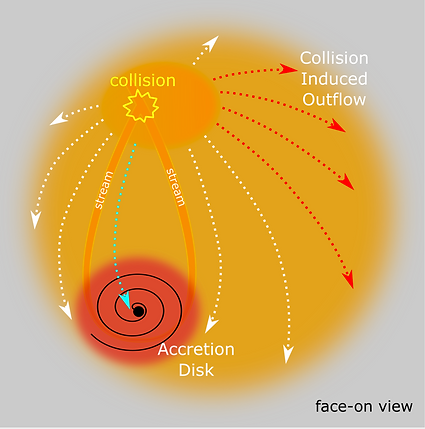
PUBLICATIONS
FAST RADIO BURSTS
[14*] Lu, W., Beniamini, P., & Kumar, P., 2021, Implications of a rapidly varying FRB in a globular cluster of M81, MNRAS accepted, PDF;
[13*] Lu, W., Kumar, P., & Zhang, B., 2020, A unified picture of Galactic and cosmological fast radio bursts, MNRAS, 498, 1397, PDF;
[12] Andreoni, I., Lu, W., Smith, R. M., et al. 2020, Zwicky Transient Facility constraints on the optical emission from the nearby repeating FRB 180916.J0158+65, ApJL, 896L, 2, PDF;
[11] Chen, G., Ravi, V., & Lu, W., 2020, The multiwavelength counterparts of fast radio bursts, ApJ, 897, 146, PDF;
[10] Lu, W., Piro, A. L., & Waxman, E., 2020, Implications of CHIME repeating FRBs, MNRAS, 498, 1973, PDF;
[9] Kumar, P., & Lu, W., 2020, Radiation Forces Constrain the FRB Mechanism, MNRAS, 494, 1217, PDF;
[8*] Lu, W., & Phinney, E. S., 2019, Imprint of local environment on fast radio burst observations, MNRAS, 496, 3308, PDF;
[7*] Lu, W., & Piro, A. L., 2019, Implications from ASKAP fast radio bursts statistics, ApJ, 883, 40, PDF;
[6] Lu, W., & Kumar, P., 2019, The maximum luminosity of fast radio bursts, MNRAS Letters, 483, L93, PDF;
[5*] Lu, W., Kumar, P., & Narayan, R., 2019, Fast radio burst source properties from polarization measurements, MNRAS, 483, 395, PDF;
[4*] Lu, W., & Kumar, P., 2018, On the radiation mechanism of repeating fast radio bursts, MNRAS, 477, 2470, PDF;
[3] Dai, L., Lu, W., 2017, Probing motion of fast radio burst sources by timing strongly lensed repeaters, ApJ, 847, 19, PDF;
[2*] Kumar, P., Lu, W., & Bhattacharya, M., 2017, Constraints on fast radio bursts source properties, MNRAS, 468, 2726, PDF;
[1] Lu, W. & Kumar, P., 2016, A universal energy distribution function for repeating fast radio bursts?, MNRAS Letters, 461, L122, PDF
TIDAL DISRUPTION EVENTS
[14] Bonnerot, C., Pessah, M. E., Lu, W., From pericenter and back: Fuller debris stream evolution in tidal disruption events, ApJL, submitted, PDF;
[13*] Batra, G., Lu, W., Bonnerot, C., Phinney, E. S., General relativistic stream crossing in tidal disruption events, MNRAS, submitted, PDF;
[12] Bonnerot, C., Lu, W., 2021, Nozzle shock in tidal disruption events, MNRAS, accepted, PDF;
[11*] Bonnerot, C., Lu, W. & Hopkins, P. F., 2021, First light from tidal disruption events, MNRAS 504, 4885, PDF;
[10] Kremer, K., Lu, W., Piro A. L., Chatterjee, S., Rasio, F. A., & Ye, C. S., 2020, Fast optical transients from stellar-mass black hole tidal disruption events in young star clusters, ApJ, 911, 104, PDF;
[9] De Colle, F. & Lu, W., 2020, Jets from tidal disruption events, New Astronomy Reviews, 89, 101538, PDF;
[8*] Bonnerot, C. & Lu, W., 2020, Simulating realistic disk formation in tidal disruption events, MNRAS, 495, 1374, PDF;
[7*] Lu, W. & Bonnerot, C. 2020, Self-intersection of the fallback stream in tidal disruption events, MNRAS, 492, 686, PDF;
[6] Kremer, K., Lu, W., Rodriguez, C. L., Lachat, M., Rasio, F. A., 2019, Tidal disruption of stars by black hole remnants in dense star clusters, ApJ, 881, 75, PDF;
[5] Lu, W. & Kumar, P., 2018, On the missing energy puzzle of tidal disruption events, ApJ, 865, 128, PDF;
[4] Lu, W., Krolik, J., Crumley, P., & Kumar, P., 2017, Radiative interaction between the relativistic jet and optically thick envelope in tidal disruption events, MNRAS, 471, 1141, PDF;
[3] Crumley, P. , Lu, W., Hernandez, R. A., Santana, R., Markoff, S., & Kumar, P., 2016, Swift J1644+57: an Ideal Test Bed of Radiation Mechanisms in a Relativistic Super-Eddington Jet, MNRAS, 460, 396, PDF;
[2] Lu, W. & Kumar, P., 2016, External inverse-Compton emission from jetted tidal disruption events, MNRAS, 458, 1071, PDF;
[1*] Lu, W., Kumar, P. & Evans, N. J., 2016, Infrared emission from tidal disruption events — probing the pc-scale dust content around galactic nuclei, MNRAS, 458, 575, PDF
BINARY BLACK HOLE FORMATION HISTORY
[1*] Lu, W., Beniamini, P. & Bonnerot, C., 2021, On the formation of GW190814, MNRAS, 500, 1817, PDF
NATURE OF BLACK HOLES
[2] Carballo-Rubio, R., Kumar, P., & Lu, W., 2018, Seeking observational evidence for the formation of trapping horizons in astrophysical black holes, PRD, 97, 123012, PDF;
[1*] Lu, W., Kumar, P. & Narayan, R., 2017, Stellar disruption events support the existence of the black hole event horizon, MNRAS, 468, 910, PDF
GAMMA-RAY BURSTS
[*4] Lu, W., Beniamini, P., & McDowell, A., 2020, Deceleration of relativistic jets with lateral expansion, MNRAS submitted, PDF;
[3] De Colle, F., Lu, W., Kumar, P., Remirez-Ruiz, E., & Smoot, G., 2018, Thermal and non-thermal emission from the cocoon of a gamma-ray burst jet, MNRAS, 478, 4553, PDF;
[2] Bhattacharya, M., Lu, W., Santana, R., & Kumar, P., 2017, Monte Carlo simulations of photospheric emission in relativistic outflows, ApJ, 852, 24, PDF;
[1] Lu, W., Kumar, P. & Smoot, G. F., 2015, Probing massive stars around gamma-ray burst progenitors, MNRAS, 453, 1458, PDF
(my favorite ones marked by *)
OTHER TRANSIENTS
[1] Piro, A. L., & Lu, W., 2020, Wind-reprocessed transients, ApJ, 894, 2, PDF
HYPER-VELOCITY STARS
[1*] Lu, W., Fuller, J., Raveh, Y., Perets, H., Li, T. S., Hosek, M. W., & Do, T., 2021, The former companion of the hyper-velocity star S5-HVS1, MNRAS, 503, 603, PDF;
OTHER ACTIVITIES
ROCK CLIMBING
I often travel to beautiful places to climb rocks, including Red River Gorge (KY), Hueco Tanks (TX), Yangshuo (China), Red Rock Canyon (NV), New River Gorge (WV), Stone Fort (TN), Yosemite (CA), Bishop (CA), etc.
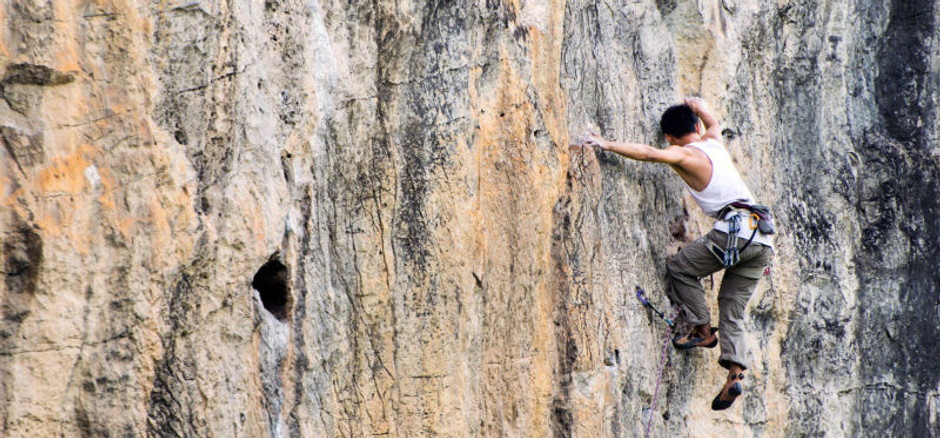
CONTACT INFORMATION

Wenbin Lu
Department of Astronomy
501 Campbell Hall
University of California at Berkeley
Berkeley, CA 94720, USA
Email: wenbinlu@berkeley.edu
Phone: +1 626-395-6829